Pathways For Carbon-Negative Biomass Fuels
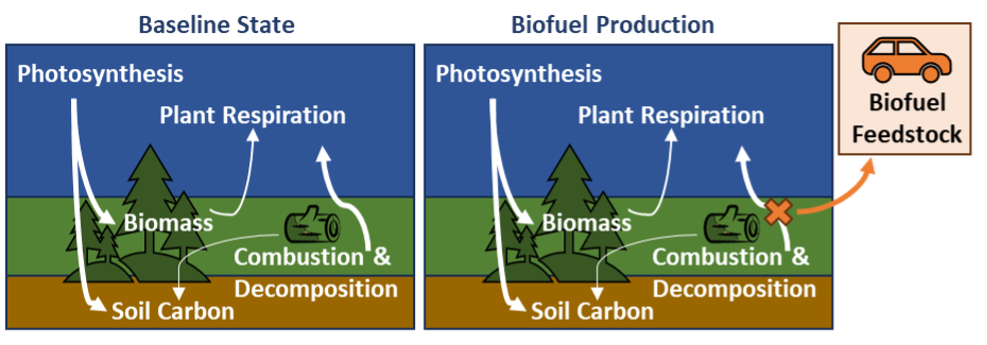
California generates millions of tons of wood waste from its farms and forests annually, but less than 20% is repurposed for commercial use. The majority is left to decay in place or is burned, contributing to greenhouse gas (GHG) emissions and air pollution. Wildfire prevention efforts, which aim to reduce biomass fuel loads on one million acres of land each year, will exacerbate the state’s wood waste problem.
Converting wood waste into biofuels can reduce overall emissions to the atmosphere. Utilization of biomass residues would not only avoid the negative impacts of current disposal practices, but also drive rural economic development, technological innovation and further emissions reductions by replacing fossil fuels.
Renewable fuels such as hydrogen, biomethane, ethanol and sustainable aviation fuel are promising options for replacing conventional transportation fuels and reducing CO2 emissions. Adding carbon capture and storage (CCS) to these fuel production facilities can provide even greater carbon dioxide removal. Coproducing biochar can further reduce the emissions impact of a biofuel system. Another approach involves the utilization of lignin to produce biomaterials or use as a petroleum bitumen substitute.
Previous studies have explored the life cycle carbon intensity (CI) of various biomass-to-biofuel pathways, encompassing diverse feedstocks, technologies and end products, such as including wood waste to RNG via anaerobic digestion, biomass to electricity via pyrolysis, and woody biomass to sustainable aviation fuel via gasification and Fisher-Tropsch synthesis.
System Boundaries
Quantifying the CI or the amount of CO2 emissions per megajoule of a biofuel involves a comprehensive approach known as life cycle assessment (LCA). LCA evaluates the environmental impact of a product or process across its entire life cycle, from the extraction of raw materials to its eventual disposal.
To gauge the CI of biofuels, an LCA begins by establishing baseline data and cataloging the energy and materials consumption of all involved processes, including carbon capture, transportation, storage and monitoring. Subsequently, they calculate the corresponding GHG emissions released into the environment. Finally, they assess the cumulative environmental effects within predefined system boundaries stemming from the biofuel system. In the case of a waste and residual biomass-to-biofuel system, system boundaries include biomass production and collection, including direct and indirect land use change; transportation of biomass to the facility; biomass preparation, including biomass chipping or grinding; biofuel production; CCS at biofuel production site; coproducts, such a biochar; fuel combustion in vehicle.
In some cases, if the biomass were to be transported to an alternate disposal site in the baseline, the net difference for the transportation to the facility may be compared to the baseline and accounted for.
Carbon Intensity Calculations
To calculate the net CO2 emissions from a biomass-to-biofuel system, an LCA baseline must be established, which is a comparison of the greenhouse gas emissions from the biofuel project to the emissions from the biomass’ fate in the absence of the project. For example, if the biomass were left to decompose in the field, the baseline scenario would include the emissions from methane production. The timing of emissions in the LCA would also need consideration. For example, if the biomass decomposes over time, we should consider the cumulative emissions from the biomass over its lifetime. However, if the biomass decomposes quickly or combusts, we can safely ignore the timing of emissions.
For this pathway example, we will consider only feedstocks that would have otherwise combusted, such as wildfire abatement residues or agricultural residues that would have been disposed of in burn piles. When biomass is burned, all the carbon that was sequestered is released into the atmosphere over a short period of time. This can be modeled as a single time pulse. The biogenic carbon released during burning is equal to the biogenic carbon that would be released from the biofuel during vehicle combustion. Therefore, the emissions from avoided burning and vehicle combustion cancel each other out, and the feedstock can be considered biogenic carbon neutral.
GHG Analysis
The GREET model considers various woody biomass feedstocks, such as forest residue and farmed trees. The life cycle GHG emissions for forest residue to FT diesel are shown in Figure 1, with two different accounting systems.
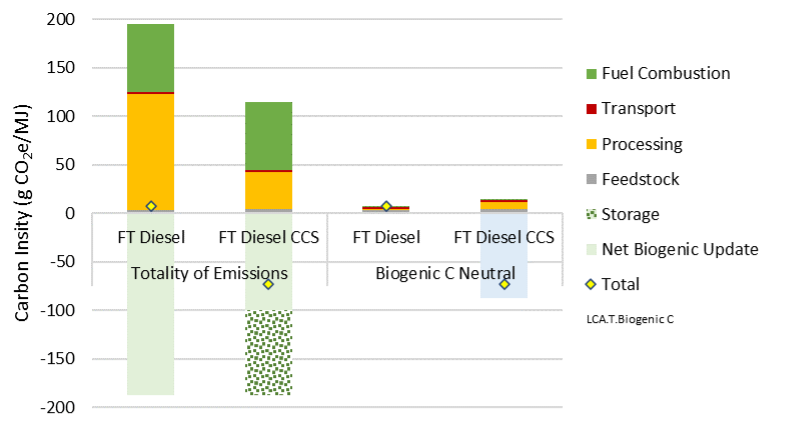
First, all the carbon flows are shown, including the net biogenic uptake and CO2 released from the process. In the pathway without CCS, process emissions plus fuel combustion equal the biogenic carbon into the process. GREET treats the net biogenic carbon flow as neutral, assuming that removal and additional growth balance. The RFS also requires that forest thinnings used for biofuel production result in increased growth of surrounding trees. When CO2 from processing emissions is stored, the net emissions are reduced. The biogenic process emissions are no longer emitted, and the net uptake results in a credit.
Identical results are achieved with a biogenic carbon-neutral accounting system. The biogenic uptake credit is omitted, and stored CO2 is treated as a credit. The latter accounting system is represented for the well-to-tank emissions in the GREET model. CCS represents a significant fraction of the CI reduction and results in a very low CI. The extent of CCS is variable with the proposed process. For example, a lower level of CO2 storage could be achieved if only concentrated CO2 sources are captured. This approach would simplify CO2 recovery efforts. Also, grid power could be used to operate equipment. Both process changes would affect system complexity, cost and GHG emissions.
The model is configured with a range of conversion pathways including gasification, pyrolysis and fermentation technologies. It examines numerous fuel pathways including hydrogen, FT diesel and jet, pyrolysis fuels, renewable natural gas and ethanol. GREET explicitly models CCS for several fuel pathways and treats the storage of organic residue from pyrolysis and anaerobic digestion as a storage credit. All the CO2 storage options provide a route for a carbon-negative pathway whether CO2 is stored as a gas, soil additive or other product. The factors influencing life cycle GHG emissions encompass energy inputs, yields and carbon storage strategies for biomass-to-fuel conversion technologies.
Figure 2 presents a range of carbon-negative technologies, offering a basis for evaluating the impact of biomass conversion to fuels. Each technology includes parameters such as biomass-to-fuel yield, power consumption, natural gas consumption, carbon storage technology and carbon capture efficiency. The default approach in the GREET model accounts for Fischer-Tropsch conversion without any carbon storage. Other cases examined include gasification, pyrolysis and fermentation technologies. These methods produce a spectrum of fuels with varying strategies for carbon storage.
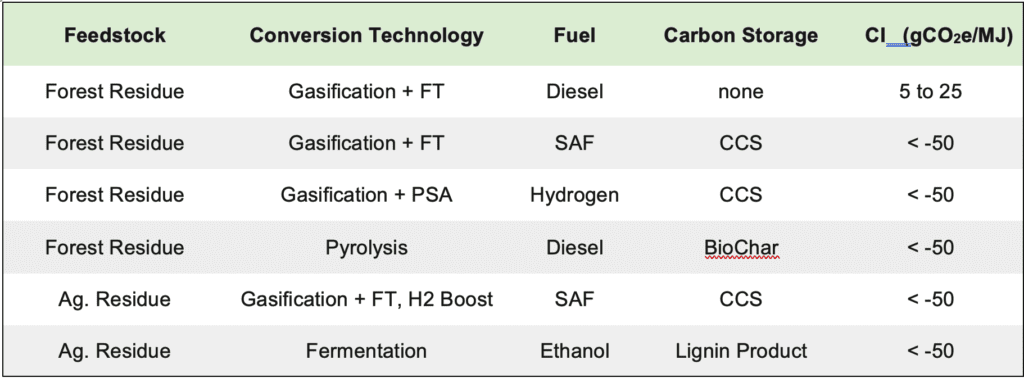
The information presented in this table draws from an array of sources to provide comprehensive background details. These sources include the GREET model, ongoing project announcements, and scientific literature.
The article was originally published in Biomass Magazine.
Prepared by Anna Redmond and Stefan Unnasch | Life Cycle Associates